Optical Fiber Communications Ever since ancient times, people had a principal need to communicate with one another. This need created interests in devising communication systems for sending messages from one distant place to another. Optical communication methods were of special interest among the many systems that people tried to use. One of the earliest known optical transmission links was a fire-signal method used by the Greeks in the eighth century BC for sending alarms, calls for help, or announcements of certain events.
Improvements of these optical transmission systems were not pursued very actively because of technology limitations at the time. For example, the speed of sending information over the communication link was limited since the transmission rate depended on how fast the senders could move their hands, the optical signal receiver was the error-prone human eye, line-of-sight transmission paths were required, and atmospheric effects such as fog and rain made the transmission path unreliable. Thus it turned out to be faster, more efficient, and more dependable to send messages by a courier over the road network.
Subsequently, no significant advances for optical communications appeared until the invention of the laser in the early 1960s and a scries of technology developments related to optical fibers around 1970. These events finally allowed practical lightwave communication systems to start being fielded worldwide in 1978. These systems operate in the near-infrared region of the electromagnetic spectrum and use optical fiber communications as the transmission medium.
The goal of this book is to describe the various technologies, implementation methodologies, and performance measurement techniques that make optical fiber communication systems possible. The reader can find additional information on the theory of light propagation in fibers, the design of links and networks, and the evolution of optical fibers, photonic devices, and optical fiber communication systems in à variety of books and conference proceedings.
This chapter gives an overview of fundamental communications concepts and illustrates how optical fiber transmission systems operate. First, gives the motivations behind developing optical fiber transmission systems. Next Sec. 1.2 defines the different spectral bands which describe various operational wavelength regions used in optical communications. Before section explains fundamental data communication concepts, encoding methods, channel capacity, and the decibel notation for expressing optical power levels. Section 1.4 gives the basic hierarchy for multiplexing digitized information streams used on optical links and Sec. 1.5 describes how wavelength division multiplexing can boost the
transmission capacity of an optical fiber significantly. Next, Sec. 1.6 introduces the functions and implementation considerations of the key elements used in optical fiber systems.
An important aspect of realizing a smoothly interacting worldwide lightwave network is to have well-established international standards for all aspects of components and networks. Section 1.7 discuss the organizations that are involved with this standardization activity and lists the main classes of standard. related to optical communication components, system operations, and installation procedures. Finally Sec. 1.8 gives an introduction to modelling and simulation tools that have been developed to aid in the design of optical fibers, passive and active devices, links, and networks.
Chapters 2 through 10 describe the purpose and performance characteristics of the major elements in an optical link. These elements include optical fibers, light sources, photodetectors, passive optical devices, optical amplifiers, and active optoelectronic devices used in multiple-wavelength networks. Then Chapters 11 through 14 show how the elements are put together to form links and networks, and explain measurement methodologies used to evaluate the performance of lightwave components and links.
Motivations for Lightwave Optical Fiber Communications
The Path to Optical Networks
Prior to about 1980 most communication technologies involved some type of electrical transmission mechanism. The era of electrical communications started in 1837 with the invention of the telegraph by Samuel F. B. Morse. The telegraph system used the Morse code, Optical Fiber Communications which represents letters and numbers by a coded series of dots and dashes. The encoded symbols were conveyed by sending short and long pulses of electricity over a copper wire at a rate of tens of pulses per second. More advanced telegraph schemes, such as the Baudot system invented in 1874, enabled the information speeds to increase to about 120 bits per second (b/s), but required the use of skilled operators. Shortly thereafter in 1876 Alexander Graham Bell developed a fundamentally different device that could transmit the entire voice signal in an analog form and which did not require any expertise to use.
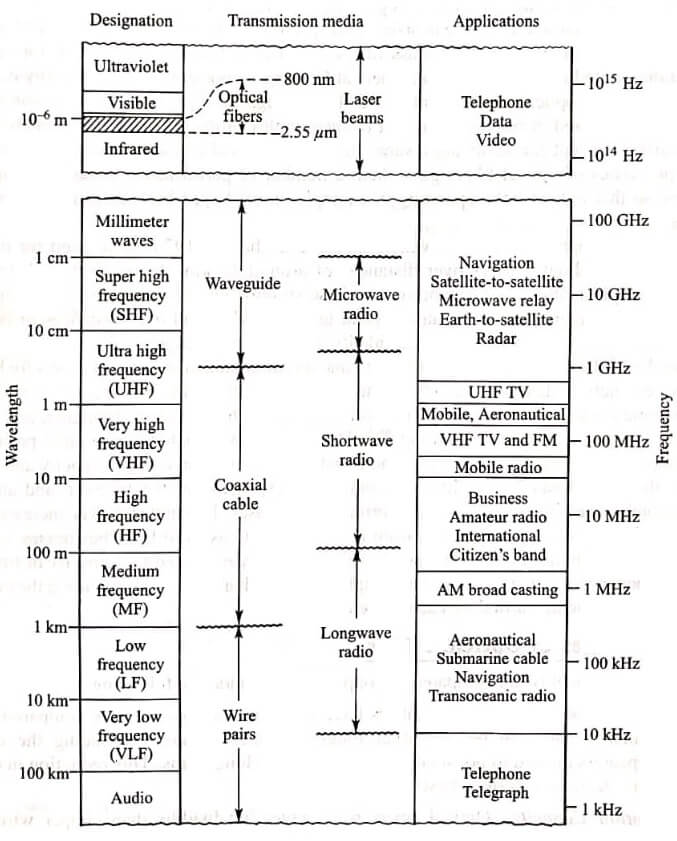
Both the telegraph and the analog voice signals were sent using a baseband transmission mode. Baseband refers to the technology in which a signal is transmitted directly over a channel. For example, this method is used on standard twisted-pair wire links running from an analog telephone to the nearest switching interface equipment. The same baseband method is used widely in optical communications, that is, the optical output from a light source is turned on and off in response to the variations in voltage levels of an information-bearing electrical signal.
In the ensuing years an increasingly larger portion of the electromagnetic spectrum was utilized to develop and deploy progressively more sophisticated and reliable electrical communication systems with larger capacities for conveying information from one place to another. The basic motivations behind each new system application were to improve the transmission fidelity so that fewer distortions or errors occur in the received message, to increase the data rate or capacity of a communication link so that more information can be sent, or to increase the transmission distance between in-line repeater or amplification stations so that messages can be sent farther without the need to restore the signal amplitude or fidelity periodically along its path. These activities led to the birth of a wide variety of communication systems that are based on using high-capacity long-distance terrestrial and undersea copper-based wire lines and wireless radio-frequency (RF), microwave, and satellite links.
In these developments the basic trend for advancing the link capacity was to use increasingly higher channel frequencies. The reason for this trend is that a time-varying baseband information-bearing
signal may be transferred over a communication channel by superimposing it onto a sinusoidal electromagnetic wave, which is known as the carrier wave or simply carrier. At the destination the baseband information signal is removed from the carrier wave and processed as desired. Since the amount of information that can be transmitted is directly related to the frequency range over which the carrier operates, increasing the carrier frequency theoretically increases the available transmission bandwidth and consequently, provides a larger information capacity.
the electromagnetic spectral bands used for radio transmission. As the diverse radio technologies move from high frequency (HF) to very high frequency (VHF) to ultra high frequency (UHF) bands with nominal carrier frequencies of 10′. 10%, and 10° Hz, respectively, increasingly higher information transmission speeds can be employed to provide a higher link capacity. Thus the trend in electrical communication system developments was to use progressively higher frequencies, which offer corresponding increases in bandwidth or information capacity.
optical frequencies are several orders of magnitude higher than those used by electrical communication systems. Thus the invention of the laser in the early 1960s aroused a curiosity about the possibility of using the optical region of the electromagnetic spectrum for transmission information. Of particular interest is the near-infrared spectral band ranging from about 770 to 1675 m since this is a low-loss region in silica glass fibers. 18-20 The technical breakthrough for optical fiber communications started in 1970 when researchers at Corning demonstrated the feasibility of producing a glass fiber having an optical power loss that was low enough for a practical transmission link.20,29
As research progressed, it became clear that many complex problems made it extremely difficult to extend the carrier concept for achieving a super broadband optical fiber communications link. Nevertheless the unique properties of optical fibers gave them a number of performance advantages compared to copper wires, so that optical links operating in a simple on-off keyed baseband mode were attractive applications.
The first installed optical fiber links which appeared in the late 1970s were used for transmitting telephony signals at about 6 Mb/s over distances of around 10 km. As research and development progressed, the sophistication and capabilities of these systems increased rapidly during the 1980s to create links carrying aggregated data rates beyond terabits per second over distances of hundreds of kilometers without the need to restore signal fidelity along the path length.
Starting in the 1990s there was a burgeoning demand on optical fiber communications -network assets for bandwidth- hungry services such as database queries, home shopping, high-definition interactive video, remote education, telemedicine and e-health, high-resolution editing of home videos, blogging, and large-scale high-capacity e-science and Grid computing.035 This demand was fueled by the rapid proliferation of personal computers (PCs) coupled with a phenomenal increase in their storage capacity and processing capabilities, the widespread availability and continuous expansion of the Internet, and an extensive choice of remotely accessible programs and information databases.
To handle the ever-increasing demand for high-bandwidth services from ranging from home-based PC users to large businesses and research organizations, telecommunication companies worldwide greatly enhanced the capacity of fiber lines by adding more independent signal-carrying wavelengths on individual fibers and increasing the transmission speed of information being carried by each wavelength.
Advantages of Optical Fibers
The advantages of optical fibers compared to copper wires include the following:
Long Distance Transmission Optical fiber comminications have lower transmission losses compared to copper wires. Consequently data can be sent over longer distances, thereby reducing the number o intermediate repeaters needed to boost and restore signals in long spans. This reduction in equipment components decreases system cost and complexity.
Large Information Capacity Optical fibers have wider bandwidths than copper wires, so that more information can be sent over a single physical line. This property decreases the number of physical lines needed for sending a given amount of information.
Small Size and Low Weight The low weight and the small dimensions of fibers offer a distinct advantage over heavy, bulky wire cables in crowded underground city ducts or in ceiling-mounted cable trays. This feature also is of importance in aircraft, satellites, and ships where small, lightweight cables are advantageous, and in tactical military applications where large amounts of cable must be unreeled and retrieved rapidly.
Immunity to Electrical Interference An especially important feature of an optical fiber relates to the fact that it is a dielectric material, which means it does not conduct electricity. This makes optical fibers immune to the electromagnetic interference effects seen in copper wires, such as inductive pickup from other adjacent signal-carrying wires or coupling of electrical noise into the line from any type of nearby equipment.
Enhanced Safety Optical fibers offer a high degree of operational safety, since they do not have the problems of ground loops, sparks, and potentially high voltages inherent in copper lines. However, precautions with respect to laser light emissions need to be observed to prevent possible eye damage.
Increased Signal Security An optical fiber offers a high degree of data security, since the optical signal is well-confined within the fiber and an opaque coating around the fiber absorbs any signal emissions. This feature is in contrast to copper wires where electrical signals potentially could be tapped off easily. Thus fibers are attractive in applications where information security is important, such as financial, legal, government, and military systems.
Optical Spectral Bands
All telecommunication systems use some form of electromagnetic energy to transmit signals. The spectrum of electromagnetic (EM) radiation. Electromagnetic energy is a combination of Optical Fiber Communications
electrical and magnetic fields and includes power, radio waves, microwaves, infrared light, visible light ultraviolet light, x rays, and gamma rays. Each discipline takes up a portion (or band) of the electromagnetic spectrum. The fundamental nature of all radiation within this spectrum is that it can be viewed as electromagnetic waves that travel at the speed of light, which is about c= 3x 10° m/s in a vacuum. Note that the speed of light s in a material is smaller by the refractive-index factor n than the speed c in a vacuum, as Chapter 2 describes. For example, n= 1.45 for silica glass, so that the speed of light in this material is about s = 2 x 10 m/s,
The physical properties of the waves in different parts of the spectrum can be measured in several interrelated ways. These are the length of one period of the wave, the energy contained in the wave, or the oscillating frequency of the wave. Whereas electrical signal transmission tends to use frequency to designate the signal operating bands, optical communication generally uses wavelength to designate the spectral operating region and photon energy or optical power when discussing topics such as signal strength or electro-optical component performance.
As can be seen, there are three different ways to measure the physical properties of a wave in various regions in the EM spectrum. These measurement units are related by some simple equations. First of all, in a vacuum the speed of light c is equal to the wavelength A times the frequency v, so that
C = λ v
where the frequency v is measured in cycles per second or heriz (Hz). The relationship between the energy of a photon and its frequency (or wavelength) is determined by the equation known as Planck’s Law
E = hv
where the parameter h = 6.63 x 10* J-s = 4.14 x 10 eV-s is Planck’s constant. The unit J means joules and the unit eV stands for electron volts. In terms of wavelength (measured in units of um), the energy in electron volts is given by
E(eV) = 1.2406 / λ (µm)
Figure 1.2 shows the optical spectrum ranges from about 5 nm in the ultraviolet region to 1 mm forfar-infrared radiation. In between these limits is the 400-to-700-nm visible band, Optical fiber communications use the near-infrared spectral band ranging from nominally 770 to 1675 nm.
The International Telecommunications Union (ITU) has designated six spectral bands for use in optical fiber communications within the 1260-to-1675-nm region.” These long-wavelength band designations arose from the attenuation characteristics of optical fibers and the performance behaviour of an crbium-doped fiber amplifier (EDFA), which Chapters 3 and 10 describe, respectively. Table defines the regions which are known by the letters O, E, S, C, L, and U.
The 770-to-910 nm band is used for shorter-wavelength multimode fiber systems. Thus this region is designated as the short-wavelength or multimode fiber band. Later chapters describe the operational performance characteristics and applications of optical fibers, electro-optic components, and other passive optical devices for use in the short- and long-wavelength bands.
Fundamental Data Communication Concepts
The exchange of information between any two devices across a communication channel involves using some type of electrical or optical signal which carries this information. The channel could be a wire, radio, microwave, satellite, atmospheric infrared, or optical fiber link. Each type of channel medium has unique transmission performance characteristics associated with it, which ideally should match the properties of the signal. However, regardless of its type the medium degrades the fidelity of the transmitted signal because of an imperfect reproduction of the signal format and because of the unavoidable presence of electrical and optical noise and interference. These impairments can lead to misinterpretations of the signal by the electronics at the receiving end.
Elementary Communication Link
Shows a block diagram of an elementary communication link. The user or device where the message originates is called a source and the final receiving user or device is called the destination. The output of the information source serves as the message input to’ a transmitter. The function of the transmitter is to couple the message. onto a transmission channel in the form of a time-varying signal that matches the transfer properties of the channel. This process is known as encoding.
As the signal travels through the channel, various imperfect properties of the channel medium and of various link components induce impairments into the signal. These include electrical or optical noise effects, signal distortions, and signal attenuation. In the presence of these impairments, the function of the receiver is to extract the weakened and distorted signal from the channel, amplify it, and restore it as close as possible to its original encoded form before decoding it and passing it on to the message destination.
Analog Signals
The format of a signal is an important factor in efficiently and reliably sending the signal across : network. The signals emitted by information sources can be classified into analog and digital formats An analog signal conveys information through a continuous and smooth variation in time of a physical quantity such as optical, electrical, or acoustical intensities and frequencies. For example, voice, music electronic hum, and video information streams are well-known analog signals.
A fundamental analog signal is the periodic sine wave, shown in Fig. 1.5. Its three main characteristics are its amplitude, period or frequency, and phase. The amplitude is the size or magnitude of the waveform
Amplitude is designated by the symbol A and is measured in volts, amperes, or watts, depending on the signal type. The frequency (designated by f) is the number of cycles per second that the wave undergoes (i.e., the number of times it oscillates per second), which is expressed in units of hertz (Hz). A hert refers to a complete cycle of the wave. The period of a wave (represented by the symbol 7) is the inverse of the frequency, that is period =T = 1/f. The term phase (designated by the symbol ø) describes the position of the waveform relative to time zero. Phase is measured in degrees or radians, where 180 degrees = π radians.
If the crests and troughs (high and low points) of two waves with identical periods are aligned, they ar said to be in phase. For example, wave 1 and wave 2 shown in Fig. 1.6 are in phase. Let wave 1 have
Example 1.1 A sine wave has a frequency f= 100kHz. Its period is T = /10° s = 0.01 ms. A sine wave has period T = 1 ns. Its frequency is f = 1/(10 s) = 1 GHz. A sine wave is offset by 1/4 of a cycle with respect time zero. since one cycle if 360 degrees, the phase shift is O = 0.25 x 360 degrees = 90 degrees = π/2 radian
amplitude A1 and let wave 2 have an amplitude A2. If these two waves are added together, the amplitude A of the resulting wave will be the sum A = A, + A. This effect is known as constructive interference.
Illustrates four phase shifts of a wave relative to time zero. When two waves differ slightly in their relative positions, they are said to be out of phase. As an illustration, the wave shown in given image (c) is 180° (7 radians) out of phase with the wave shown in Fig. 1.7(a). If the two waves in image (a) and 1.7(c) have identical periods, the resulting wave amplitude will be the sum A = A, + A,. If the two waves also have the same amplitudes, then they undergo destructive interference so that A =0, that is they will cancel each other out. These interference concepts are important when considering the operation of devices such as laser diodes, thin-film filters, and optical couplers.
Example 1.2 If the spectrum of a signal ranges from its lowest frequency flow = 10 kHz to its highest frequency
fhigh = 100 kHz, then the bandwidth B= fhigh – flow = 90 kHz,
Two further common characteristics in communications are the frequency spectrum (r simply spectrum) and the bandwidth of a signal. The spectrum of a signal is the range of frequencies that it contains. That is, the spectrum of a signal is the combination of all the individual sine waves of different frequencies which make up that signal. The bandwidth (designated by B) refers to the width of this spectrum. The bandwidth commonly is specified in units such as kilohertz (kHz), megahertz (MHz), or gigahertz. (GHz).
Digital Signals – Optical Fiber Communications
A digital signal is an ordered sequence of discrete symbols selected from a finite set of elements, for example, the letters of an alphabet, numbers, and other symbols such as @, #, or %. A common digital signal configuration is the binary waveform, which consists of two pulse types of known shape as shown in Fig. 1.8. The information contained in a digital signal is given by the particular sequence of the presence (a binary one, or simply either one or 1) and absence (a binary zero, or simply either zeroor 0) of these pulses or bits. These bits often are referred to as a logic one (or logic 1) and a logic zera (or logic 0), respectively.
The time slot T, in which a bit occurs is called either the bit interval, bit period, or bit time. The bit intervals are spaced regularly and occur every 1/R seconds, or at a rate of R bits per second (b/s), where R is the bit rate or the data rate. The data rate commonly is specified in units such as kilobits per second
(kb/s), megabits per second (Mb/s), or gigabits per second (Gb/s). As an example, a data rate of 2 x 10° b/s = 2 Gb/s. A bit can fill the entire bit interval or part of it, respectively. A block of eight bits often is uscd to represent an encoded symbol or word and is called an octet or a byte.
Digitization of Analog Signals
To send a signal in an analog format, the transmission channel typically cannot achieve perfect reproduction of the signal at the destination, so there always will be some degree of distortion. Furthermore, different types of analog signals may require different channel responses. This puts a strain on the design of a multipurpose analog link. In addition, noises that get added to the analog signal as it passes through multiple repeaters cannot be eliminated, which further degrades the signal.
In contrast, digital signals can undergo a great amount of distortion and still allow the information to be extracted with a high degree of fidelity. To avoid the shortcomings of analog signals and to create networks that can multiplex and switch any type of information, most information is sent in a digital format. To achieve this network flexibility, most analog signals need to be converted to a digital format. However, as Chapter 9 describes, there are a number of situations in which it is advantageous to send high- speed analog signals in their native form over relatively short distances. One example is the transmission of microwave signals from a satellite dish to a processing station located less than a kilometer away.
An analog signal can be transformed into a digital signal through a process of periodic sampling and the assignment of quantized values to represent the intensity of the signal at regular intervals of time. To convert an analog signal to a digital form, one starts by taking instantaneous measures of the height of the signal wave at regular intervals, which is called sampling the signal. The simplest, but not necessarily the best, way to convert these analog samples to a digital format is to divide the amplitude excursion of the analog signal into N equally spaced levels and assign a discrete binary word to each level. Each analog sample is then assigned one of these level values. This process is known as quantization. Since the signal varies continuously in time, this process generates a sequence of real numbers.
Before shows an example of digitization. Here the allowed voltage-amplitude excursion is divided into eight equally spaced levels ranging from zero to V volts. In this figure, samples are taken every microsecond and the nearest discrete quantization level is chosen as the one to be transmitted, according to the 3-bit binary code listed next to the quantized levels shown in before
At the receiver this digital signal is then demodulated. That is, the quantized levels are reassembled into a continuously varying analog waveform.
Network Information Rates
To handle the continuously rising demand for high-bandwidth services from users ranging from individuals to large businesses and research organizations, telecommunication companies worldwide are implementing increasingly sophisticated digital multiplexing techniques that allow a larger number of independent information streams to share the same physical transmission channel. This section describes some common digital signal multiplexing techniques.
Telecom Signal Multiplexing
Table gives examples of information rates for some typical telecom services. To send these services from one user to another, network providers combine the signals from many different users and send the aggregate signal over a single transmission line. This scheme is known as time-division-multiplexing (TDM) wherein N independent information streams, each running at a data rate of R b/s, are interleaved electrically into a single information stream operating at a higher rate of N x R b/s. To get a detailed perspective of this methodology, let us look at the multiplexing schemes used in telecommunications.
Early applications of fiber optic transmission links were mainly for large capacity telephone lines. These digital links consisted of time-division-multiplexed 64-kbls voice channels. The multiplexing was developed in the 1960s and is based on what is known as the plesiochronous digital hierarchy (PDH). Figure 1.13 shows the digital transmission hierarchy used in the North American telephone network.
The fundamental building block is a 1.544-Mb/s transmission rate known as a DS1 rate, where DS stands for digital system. It is formed by time-division-multiplexing twenty-four voice channels, each digitized at a 64-kb/s rate (which is referred to as DSO). Framing bits, which indicate where an information unit starts and ends, are added along with these voice channels to yield the 1.544-Mbls bit stream. Framing and other control bits that may get added to an information unit in a digital stream are called overhead bits. At any multiplexing level a signal at the designated input rate is combined with other input signals at the same rate.
elements such as Pr. Th, and Er creates optical fiber amplifiers (PDFA, TDFA, and EDFA devices). These devices and the use of Raman amplification gave a further capacity boost to long-wavelength WDM system. Optical Fiber Communications
Special material-purification processes can eliminate almost all water molecules from the glass fiber matenal, thereby dramatically reducing the water-attenuation peak around 1400 nm. This process opens the E-band (1360-to-1460 nm) transmission region to provide around 100 nm more spectral bandwidth in these specially fabricated fibers than in conventional single-mode fibers.
Systems operating at 1550 nm provide the lowest atenuation, but the signal dispersion in. silica fiber is larger at 1550 nm than at 1310 nm. Fiber manufacturers overcame this limitation first by standard eating the dispersion-shifted fibers for single-wavelength operation and then by devising non-zero dispersion-shifted fiber (NZDSF) for use with WDM implementations.
The latter fiber type bo the widespread use of multiple-wavelength S-band and C-band systems for high-capacity, Jone to terrestrial and undersea transmission links. These links routinely carry traffic at 10 Gb/s (OC-192s 64) over nominally 90-km distances between amplifiers or repeaters. By 2005 links operating at 40 G were being installed and field trials of 160-Gb/s long-distance transmission systems were t successfully.9-42
Standards for Optical Fiber Communications
To allow components and equipment from different vendors to interface with one another, numerous international standards have been developed.*5-45 The three basic classes for fiber optics are primary standards, component testing standards, and system standards.
Primary standards
Refer to measuring and characterizing fundamental physical parameters such as attenuation, bandwidth, operational characteristics of fibers, and optical power levels and spectral widths. In the USA the main organization involved in primary standards is the National Institute of Standards and Technology (NIST). This organization carries out fiber optic and laser standardization work, and it sponsors an annual conference on optical fiber measurements. Other national organizations include the National Physical Laboratory (NPL) in the United Kingdom and the Physikalisch-Technische Bundesanstalt (PTB) in Germany. Optical Fiber Communications
Component testing standards
Define tests for fiber-optic component performance and they establish equipment-calibration procedures. Several different organizations are involved in formulating testing standards, some very active ones being the Telecommunications Industry Association (TIA) in association with the Electronics Industries Alliance (EIA), the Telecommunication Sector of the International Telecommunication Union (ITU-T), and the International Electrotechnical Commission (IEC).
The TIA has a list of over 120 fiber optic test standards and specifications under the genera designation TIA/EIA-455-XX-YY, where XX refers to a specific measurement technique and YY refers to the publication year. These standards are also called Fiber Optic Test Procedures (FOTP), so ha TIA/EIA-455-XX becomes FOTP-XX. These include a wide variety of recommended methods for testing the response of fibers, cables, passive devices, and electro-optic components to environmental factors and operational conditions. For example, TIA/EIA-455-60-1997, or FOTP-60, is a method published 1997 for measuring fiber or cable length.